Keywords
|
Pulse detonation engine, Fire Control System, Data acquisition, Thrust / Pressure measurement & Instrumentation |
INTRODUCTION
|
A pulse detonation engine, or "PDE", is a type of propulsion system that uses detonation waves to combust the fuel and oxidizer mixture. The engine is pulsed because the mixture must be renewed in the combustion chamber between each detonation wave initiated by an ignition source. Theoretically, a PDE can operate from subsonic up to a hypersonic flight speed of roughly Mach 5. An ideal PDE design can have a thermodynamic efficiency higher than other designs like turbojets and turbofans because a detonation wave rapidly compresses the mixture and adds heat at constant volume. Consequently, moving parts like compressor spools are not necessarily required in the engine, which could significantly reduce overall weight and cost. PDEs have been considered for propulsion for over 70 years. Key issues for further development include fast and efficient mixing of the fuel and oxidizer, the prevention of auto-ignition, and integration with an inlet and nozzle. |
The Paper is organized in following Paras: Concept of PDE, Detonation Initiation, Design of Pulse detonation for PEC Experimental Setup, Pulse Detonation Engine Details, Fuel Injection/Air Delivery, Channel Calibration/ Voltage-to-Thrust Conversion, Test Cell / PDE Control, Data Acquisition, Challenges faced including their remedial Management, Results, Data Extraction, Data Accuracy, Achievements from the project and future course of work is covered. It is followed by acknowledgements and references too. |
CONCEPT OF PDE
|
All regular jet engines and most rocket engines operate on the deflagration of fuel, that is, the rapid but subsonic combustion of fuel. The pulse detonation engine is a concept currently in active development to create a jet engine that operates on the supersonic detonation of fuel. The basic operation of the PDE is similar to that of the pulse jet engine; air is mixed with fuel to create a flammable mixture that is then ignited. The resulting combustion greatly increases the pressure of the mixture to approximately 100 atmospheres (10 MPa), which then expands through a nozzle for thrust. To ensure that the mixture exits to the rear, thereby pushing the aircraft forward, a series of shutters are used to close off the front of the engine. Careful tuning of the inlet ensures the shutters close at the right time to force the air to travel in one direction only through the engine. The main difference between a PDE and a traditional pulse jet engine is that the mixture does not undergo subsonic combustion but instead, supersonic detonation. In the PDE, the oxygen and fuel combination process is supersonic, effectively an explosion instead of burning. The other difference is that the shutters are replaced by more sophisticated valves. In some PDE designs from General Electric, the shutters are eliminated through careful timing, using the pressure differences between the different areas of the engine to ensure the "shot" is ejected rearward. The main side effect of the change in cycle is that the PDE is considerably more efficient. In the pulse jet engine the combustion pushes a considerable amount of the fuel/air mix (the charge) out the rear of the engine before it has had a chance to burn (thus the trail of flame seen on the V-1 flying bomb). Even while inside the engine the mixture's volume is continually changing, which is an inefficient way to burn fuel. In contrast, the PDE deliberately uses a high-speed combustion process that burns all of the charge while it is still inside the engine at a constant volume. This is said to increase the amount of heat produced per unit of fuel above any other engines, although conversion of that energy into thrust would remain inefficient. A combustion process able to produce more heat per unit of fuel would, of course, be incredibly valuable in countless applications. Another side effect, not yet demonstrated in practical use, is the cycle time. A traditional pulsejet tops out at about 250 pulses per second due to the cycle time of the mechanical shutters, but the aim of the PDE is thousands of pulses per second, so fast that it is basically continuous from an engineering perspective. This should help smooth out the otherwise highly vibrational pulsejet engine — many small pulses will create less volume than a smaller number of larger pulses for the same net thrust. Unfortunately, detonations are many times louder than deflagrations. The major difficulty with a pulse-detonation engine is starting the detonation. While it is possible to start a detonation directly with a large spark, the amount of energy input is very large and is not practical for an engine. The typical solution is to use a deflagration-todetonation transition (DDT)—that is, start a high-energy deflagration, and have it accelerate down a tube to the point where it becomes fast enough to become a detonation. Alternatively the detonation can be sent around a circle and valves ensure that only the highest peak power can leak into exhaust. This process is far more complicated than it sounds, due to the resistance the advancing wave front encounters (similar to wave drag). DDTs occur far more readily if there are obstacles in the tube. The most widely used is the "Shchelkin spiral", which is designed to create the most useful eddies with the least resistance to the moving fuel/air/exhaust mixture. The eddies lead to the flame separating into multiple fronts, some of which go backwards and collide with other fronts, and then accelerate into fronts ahead of them. The behaviour is difficult to model and to predict, and research is on-going. As with conventional pulsejets, there are two main types of designs: valved and valveless. Designs with valves encounter the same difficult-to-resolve wear issues encountered with their pulsejet equivalents. Valveless designs typically rely on abnormalities in the air flow to ensure a one-way flow, and are very hard to achieve in regular DDT. NASA maintains a research program on the PDE, which is aimed at high-speed, about Mach 5, civilian transport systems. However most PDE research is military in nature, as the engine could be used to develop a new generation of high-speed, long-range reconnaissance aircraft that would fly high enough to be out of range of any current anti-aircraft defences, while offering range considerably greater than the SR-71, which required a massive tanker support fleet to use in operation. While most research is on the high speed regime, newer designs with much higher pulse rates in the hundreds of thousands appear to work well even at subsonic speeds. Whereas traditional engine designs always include trade-offs that limit them to a "best speed" range, the PDE appears to outperform them at all speeds. Both Pratt & Whitney and General Electric now have active PDE research programs in an attempt to commercialize the designs. Key difficulties in pulse detonation engines are achieving DDT without requiring a tube long enough to make it impractical and drag-imposing on the aircraft (adding a U-bend into the tube extinguishes the detonation wave); reducing the noise (often described as sounding like a jackhammer); and damping the severe vibration caused by the operation of the engine. |
DETONATION INITIATION
|
Of critical importance in continued PDE research is the development of an efficient process with which to produce reliable, repeatable detonation waves. The minimum diameter and distance within a combustor required for detonation initiation are fundamentally limiting factors which influence both size of the engine and operating frequency range. Additionally, the carriage of onboard oxidizer will effectively negate performance gains offered by the use of PDE technology. Research continues into techniques which reduce initiation time and initiation distance. The most common techniques in the research community are direct initiation, shock focusing, and Deflagration-to-Detonation Transition (DDT). |
A. Direct Initiation: |
Direct initiation is defined by Frolov as a near-instantaneous application of energy into a fuel mixture which generates a blast wave of sufficient strength and intensity to decay into a self-sustained detonation [11]. Application of this technique would require the use of high explosives (not practical for PDE) or electrical initiation sources such as lasers or sparks. Energy levels on the order of 100-1000 kJ applied on a cyclic basis would be necessary and at this time are not feasible. This technique has the potential to offer the shortest detonation time and lengths, and remains and area of active research; however, limitations listed above require current PDE research to incorporate self-initiation techniques or those with low net power requirements. |
B. Shock Focusing: |
Shock focusing techniques use geometric designs to focus a shock wave or multiple shock waves to create regions of high temperatures and pressures which results in the local ignition of a reactive mixture. Research conducted by Channel found that multiple methods can be used to achieve focusing of these waves such as combustor geometry, turbulence devices or injection jets [10]. In a reactive mixture, these regions of high temperature can produce violent explosions, and eventually detonations. This technique is strongly dependent on both engine geometry and the initial creation of moderate shock waves, and has proven to be of limited value within the dynamic constraints of PDE research. |
DESIGN OF PULSE DETONATION FOR PEC EXPERIMENTAL SETUP
|
This section provides information specific to the equipment and techniques used to complete the experimentation performed in support of this work (figure All testing associated with this project occurred in the Aerospace Engineering Department of PEC University of Technology Chandigarh campus site [1]. An engine capable of burning both Acetylene/oxygen and Acetylene/air mixture was required to complete the desired research. A PDE designed and used for previous experimentation was used as the baseline engine for this project [10]. Prior to initial experimentation, numerous modifications were made to the engine and test cell to improve on the previous design and enable switching between fuel types with minimum impact. New fuel supply systems were constructed, a thrust stand was incorporated to measure axial thrust data, and new data acquisition software created for high-speed data capture. |
PULSE DETONATION ENGINE DETAILS
|
A single tube, “valveless” PDE was constructed by the investigating team conducting thesis research. The engine consisted of a combustion tube, fuel injector system, and ignition system. Since the initial design, modifications made by current research team to fuel injector scheme, flow choke sizing, fuel/air inlet geometry, spiral dimensions and mounting technique, tube diameter, and combustion chamber volume have been implemented to facilitate performance improvements throughout continuous design process. Specific engine parameters and systems used during experimentation in support of this research are discussed below and photograph of the test rig is included in Fig. 2. |
FUEL INJECTION/AIR DELIVERY
|
The fuel injection/air delivery system was designed to provide control of the stoichiometry of the fuel/air mixture supplied to the combustor. Supply air delivery incorporated a valveless design in that a constant flow of supply air was provided to the engine. Control of the fuel mixture was achieved by varying the pressure of injected fuel. Fuel pressure changes altered the mass flow rate ratio of fuel to supply air, which is defined as the equivalency ratio (φ). The mathematical expression for equivalence ratio is given by |
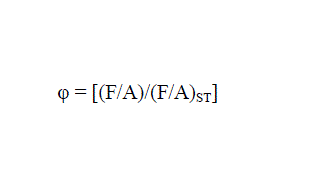 |
Where (F/A) is the mass ratio of fuel to air for the experimental mixture and (F/A)ST is the mass ratio of fuel to air for the stoichiometric mixture. Performance varied with changing equivalency (φ) ratio |
A. Oxygen/Air Delivery: |
Supply air was delivered from O2 cylinder at varying mass flow rates w.r.t. the pressure in the pipe line controlled by the valves in line. The supply was controlled by solenoid valve operated through Lab view software. |
B. Acetylene Injection: |
An independent injector system was constructed to supply Acetylene from cylinder into the combustion chamber. Electricallycontrolled high frequency Techno make solenoid valve injector was joined by a common feed manifold and mounted to the fuel arm downstream of the flow chokes. The gaseous fuel mixed with supply air prior to entry into the combustion chamber. |
C. Ignition System: |
The ignition system consists of a 24 V DC Condenser and spark plug arrangement. The control for the spark plug ignition is with the control circuit only. The VI control gives an option to ignite the spark plug after the gas has been released into the system for the required amount of time. |
The oxygen inlet and the acetylene inlet are near the spark plug itself, which enables us to start the combustion, by imparting a high voltage spark to the gases. |
D. Combustion Tube: |
The combustion tube is a hollow steel pipe (Grade 304) with an internal diameter of 48 mm and external diameter of 60 mm, providing us a thickness of 6 mm which is strong enough to withstand the detonation pressures generated inside the tube. The combustion tubes are of various sizes, 20 cm, 40 cm, 80 cm and 100 cm. These various sizes enable us to get any size tube for our experiment in the steps of 20 cm with a minimum length of 70 cm. |
E. Primary Structure: |
The primary structure comprises of the combustion tubes lying on the middle support bar. The combustion tubes are encapsulated in a curved wedge fitted on a slider, to reduce friction to the least amount possible. The fully loaded system with 3.5 m tube length has a friction of 17 N. |
F. Load Cell: |
The load cells (Max Load Make) fitted in the structure are high capacity load cells with a maximum value of 500 kgf. The loads cells are fitted on the one extreme point of the structure on the axis of the tube, which allows us to measure the thrust produced on the system. |
G. Data Collection Hardware: |
The data collection hardware comprises of 2 25 port boards, a low resistance cable of 16 metres length, a NI DAQ system, a USB carrier, 4 pressure sensors (MEAS make), 3 thermocouples, 3 load cells (Maxload Make). |
H. Engine/Thrust Stand Interface: |
Thrust stand means the test rig fabricated that will support the entire engine on it. The thrust stand is 12 feet long, 3 feet wide, 4 feet high in dimensions, with another 1.5 feet curved cowl covering over it, to prevent any mishaps to the people working nearby the structure. |
I. Thrust Stand: |
As in all systems including thrust stand, which contain mass and elasticity, the thrust stand was assumed to be capable of free vibration, as well as forced vibration at the excitation frequency which PDE testing would occur. Vibration analysis identifies the primary physical characteristics which govern system response as mass, damping and stiffness. In the presence of light (assumed here) or no damping, the system will vibrate very near the natural frequency defined by |
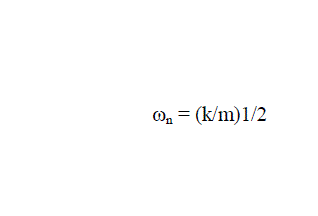 |
Where k is system stiffness and m represents system mass [17]. |
CHANNEL CALIBRATION/ VOLTAGE-TO-THRUST CONVERSION
|
The first phase of the process consisted of calibration of the individual channels which transfer load cell data to the data collection system and convert voltage data to force units. Small imperfections in cable manufacturing, varying length of supply cable per channel, and other factors lead to small variances during the data transmission process which must be normalized to eliminate constant sources of bias error. This process was completed in conjunction with manufacturer representatives, with no load on the thrust stand and support pins installed. The first step was to install a load cell simulator in place of actual load cells, and apply excitation voltages at known excitation ranges. |
TEST CELL/ PDE CONTROL
|
The engine and support equipment in test cell #2 was controlled by a National Instruments (NI) Real-Time VI software program installed on a personal computer (PC) in the control room with terminations. The PC was linked to a NI PXI-1000B controller in the test cell through an internet connection to the PXI IP address. The program controlled engine operation by managing engine supply gas operation via ball valves located in the test cell. A BNC pulse generator also located in the control room controlled timing of trigger signals sent to both the fuel injectors and TPI via electrical relays located within the test cell. Also located within the control room were 24 VDC and 220 VAC master power switches, and an emergency shutoff button. The electrical switches controlled electrical power within the cell for engine control and instrumentation equipment, such as temperature and pressure transducers connected through termination boxes. The emergency shutoff button was provided as a safety feature and would disable test cell #2 by closing all supply gas ball valves and interrupt fuel injection and ignition trigger signals. |
DATA ACQUISITION
|
Data acquisition was controlled via the PC in the control room. The PC was linked to the SCXI-1000B controller mounted on the wall in the test cell, which in turn was linked to three NI data acquisition devices (DAQ) located on the engine stand. The PXI- 6031E was 8 channel, 16 bit card which collected operational parameters such as various engine temperatures and pressures, and supply gas pressures, at a rate of 10 kHz. A data file path designated on the control program directed data in the form of an excel spreadsheet to a selected location. These data streams were used to calibrate the stand prior to the commencement of testing, and record thrust data during engine operation. A second file path designated on the control panel directed thrust data in the form of an excel spreadsheet to a selected location. Additionally, thrust data was streamed to the control program GUI for real-time monitoring. |
THE CHALLENGES FACED AND THEIR REMEDIAL MANAGEMENT
|
During the design of test rig and firing of system, a large no. of problems have cropped. All these issues were addressed as follows: |
A. Noise in all channels: |
There was a huge noise in output of DAQ resulting in signal getting attenuated (refer fig. 3). So, proper grounding, shielding and crimping was initiated to resolve the issue. |
B. Data loss: |
There was a data loss observed during testing due long cables. It was in tune of mV while travelling a distance of 35 m. This defect was confirmed through Digital multimeter readings at sensor and DAQ end. This was more than 10%, when signals were small, making it further worse. Since distance was required for safety clearances, so it canâÃâ¬ÃŸt be addressed through that route. So, problem was redressed by changing the data cable to low loss cables of resistance 0.01Ω/m with MIL connectors (refer fig. 4). |
C. Sampling Frequency Problem: |
During the PDE firing, many occasions, large portion of data was clipped due faulty double buffering schemes used for data acquiring. So, the DAQ sampling frequency was increased to > 1MS/s and problem got resolved (refer fig. 5). |
D. Accidental Firing Handling: |
Multiple trigger inter locks are designed to place the system to prevent accidental firing. These interlocks are O2 & C2H2 mechanical gate valves on cylinders, Power supply connections to igniters, 2 stage Fire control systems and multi-level strong password control on FCS make it difficult to fire accidentally. |
E. Flow Pressures: |
Analog gauges were introduced at cylinders for better safeties and enhanced ease for regulation of flow to pulse tube. (refer fig. 6). |
F. Back flow of gas: |
The back flow of gasses back to cylinders was poising grave problems of safety in the propulsion/structural lab. At higher pressures it was further worse to make it hazardous configuration. The problem redressal was carried out by fitting the Solenoid valves with Non-Return Valves (NRV) in upline near the test rig in O2 and C2H2 lines. (refer fig. 7). |
G. Firing Hazards: |
The firing of PDE in PEC campus was a serious safety hazards in vicinity of students. Proper cordoning with danger flags and following an approved finalised firing sequence was put to use. The sequence is as follow: |
The Firing Checklist:
|
i. Clear the trial area by following all necessary safety drills. Cordon off the test bed with red ribbons, flags and Safety Officers placements. |
ii. Connect all the pneumatic and electrical lines |
iii. Make the DAQ, PS and instrumentation computer ready |
iv. Check O2 & C2H2 cylinders& open mechanical valves & pressurise lines accordingly |
v. Make the count down after re-verify hardware and alert siren. |
i. Fire solenoid valves to release O2& C2H2into the pulse tube through computer based software |
ii. Fire the igniter through FCS through computer based especially designed software |
iii. Record the DAQ results in CSV format in computer using data loggers |
iv. Clear siren and release the emergency |
v. Import the Recorded data to Matlab |
vi. Plot the Graphs |
vii. Analyse the data obtained |
H. General Safety issues: |
Handling high energy gases is always dangerous. So proper safety check list created to suggest Safety stand-off distance and other vital safeties, which is as under: |
The cautions and Safeties: |
i. The stand should be properly anchored |
ii. NRV should be fitted in solenoid valve area to prevent back flow |
iii. Fire extinguishers should be placed at accessible areas |
iv. Areas should be cordoned off well before |
v. Block house should be created for launch teams |
vi. In case of any miss-fire / auto fire, a proper drill should be worked out. |
vii. All power supplies should be disconnected, when not in use. |
viii. All pneumatic supplies should be disconnected, when not in use. |
I. Rain problem: |
The test rig is kept outside in the lawns of Aerospace Engg. Department. Hot sun, rain and other environmental hazards affect the life of the system. So redressal plan was created to make all weather cover to protect the system. (refer fig. 8). |
J. Stand-off : |
As the thrust increased, so stand-off again became an issue. To make safety stand-off O2 and acetylene cylinders were kept at distance of 30 m from pulse tube. Further, Technology was used to address the problem. The desktop of FCS was taken over internet using team viewer. Firing was carried out from a remote block house. |
K. Disturbed flow: |
There was a lot of disturbance resulting in improper mixing of gases in the tube. So settling chambers (refer fig. 9). were designed and implemented to make the flow smooth resulting in better mixing and detonation successes. |
L. Gas Leaks: |
There is a chance of accidental gas leakage from hose/port connections. So to ensure safety, all the hose connections were reinforced using metal clips (refer fig. 10). with nut tights to ensure no leak at joints. |
M. Hazardous gas handling: |
As the pressures of C2H2 and O2 were increased to get more thrust, chances of rubberised metal braded hoses to give away has increased. To come out of that problem, GI piping (refer fig. 11). from Cylinder till Pulse Detonation tube was carried out. This ensured longer life alongwith rigid clean design. |
N. Flame control: |
Proposed to put a flame containment system for flame checks downstream the flow across Pulse tube. |
O. Heat handling: |
Due high temperature in tube, planned to gap of time for cooling of tube along with forced cooling at key areas with introduction of vents at high concentration zones. |
P. Cooled sensors: |
Present pressure and temperature sensors are working satisfactory under current conditions, future requirements of firing PDE in 10 HzâÃâ¬ÃŸs or above for continuous firing, cooled sensors are required to be incorporated. After incorporating vital changes, firings were carried out. |
RESULTS
|
The initial objectives of research conducted in support of this work were to experimentally measure the thrust profile of a PDE combusting both Acetylene and oxygen to incorporate an experimentally verified fuel mass flow rate to compute specific impulse values for both fuels at various firing rates. A significant effort was made to understand how to operate the engine and understand fuelling, timing and ignition effects on engine operation. Several months were spent conducting familiarization runs in preparation for data collection events, during which time suitable fuel/timing event profiles were established. As test firings began, it became immediately obvious that harmonics of the thrust stand were excited by combustion events and masked the true thrust profile. At this point, the objectives of this thesis evolved into designing an analysis system capable of filtering undesired thrust stand responses and returning accurate detonation thrust information. Additionally, system response varied with engine mounting techniques, which lead to an investigation into determining the optimal engine mounting design. A final objective of this research was to understand this phenomenon and assess its impact on the standâÃâ¬ÃŸs capability to accurately measure PDE thrust data. Raw data from the experimental runs listed above displayed a largely sinusoidal output. Actual thrust profiles from a nominal PDE should display a series of impulse thrust spikes of positive forces at the operational frequency of the engine. An average thrust profile would then be obtained by integrating the area under the thrust curve. |
As this was the first such engine the Aerospace Engineering Department of PEC University of Technology, Chandigarh independently designed filtering techniques to extract average thrust values from the raw data. Figure no. 12 & 13 depicts typical firing results in form of Pressures & thrusts plot. The results obtained from various test runs are analysed to draw the inferences. Comparative analysis is plotted below as desired. |
The Consolidated results are shown below: |
The pressures obtained are cross plotterd for comparasion purposes: |
The thrusts obtained are cross plotterd for comparasion purposes: |
DATA EXTRACTION
|
The above is the setup for data extraction. The extracted data is displayed online & saved in excel file for more detailed analysis. |
DATA ACCURACY
|
Data accuracy for the TMS/low pass filter system described above was computed using the root mean square function. The two major sources of error were introduced by the data acquisition hardware (NI DAQ) and calibration/strain gauge fidelity of the TMS. National Instruments lists on their website detailed accuracy analysis of all supported DAQ equipment. For the hardware installed on this rig, the company cites offset, system noise and temperature drift as the predominant error sources, and reports a system absolute accuracy of +/- 0.377%. |
ACHIEVEMENTS FROM THE PROJECT
|
After analysing the data, the process of experimental analysis is perfectly established. All the range of sensors was also established. The specifications of DAQ and response times for sensors are now changed. Technology of design of test rig, launch tube, helical, cabling, igniters, cylinders, piping, and wiring are established to very high level of confidence. So the project has achieved all its objectives and created an infrastructure for future research work too. |
FUTURE COURSE OF WORK
|
Having created the data and test rig, now stage is set to analyse and supplement experimentally the PDE technology. It can help in depth analysis of PDE characteristics of C2H2& O2. Other types of fuels including gaseous fuels can also be tried for comparison purposes. Fuel jets, input pressures manipulations, tube length / diameter optimisation, Shchelkin (helical) length, position change of igniters are the future key areas. Storage Oscilloscope usage, velocity & torque measurements can also be captured in due course. |
ACKNOWLEDGEMENTS
|
The team is thankful to Director, TBRL for his kind endeavour. The team also is obliged to Prof. Manoj Datta, Director of PEC University and Prof. PS Satsangi, Head, AED and AED team for their support and co-operation to the work. |
Figures at a glance
|
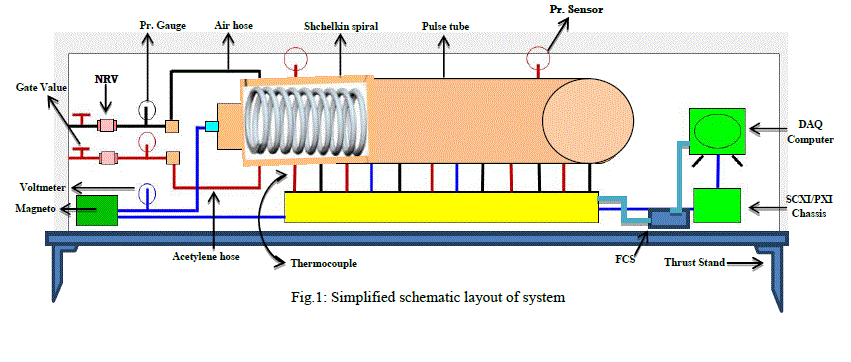 |
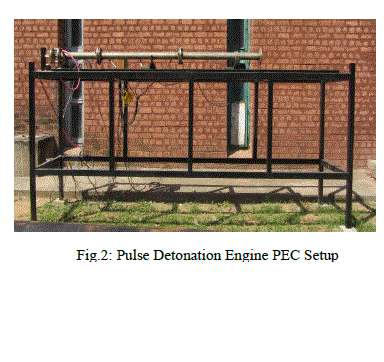 |
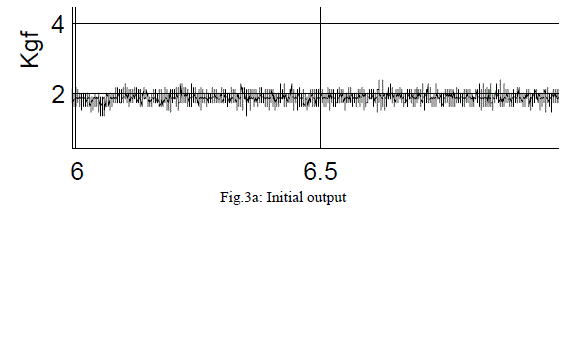 |
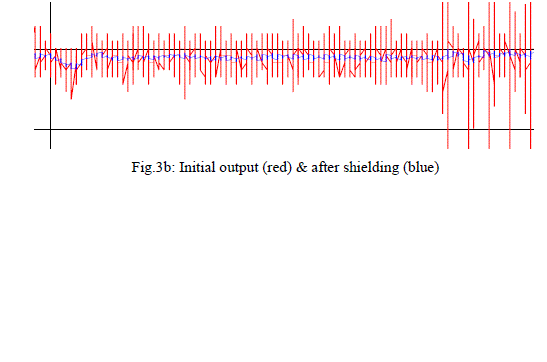 |
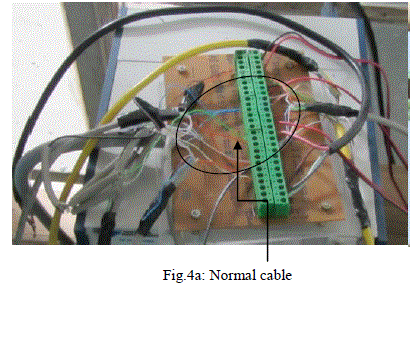 |
Figure 1 |
Figure 2 |
Figure 3a |
Figure 3b |
Figure 4a |
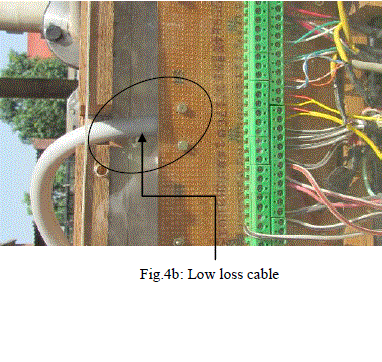 |
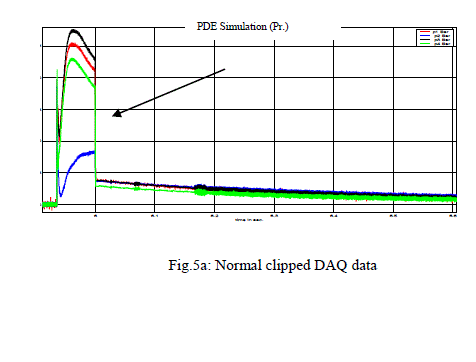 |
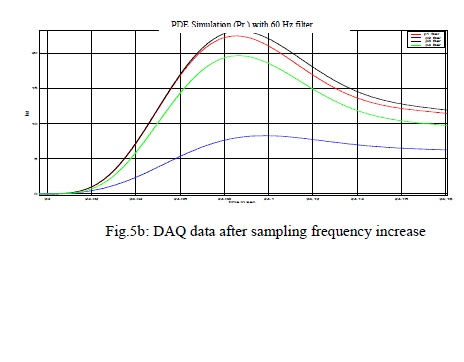 |
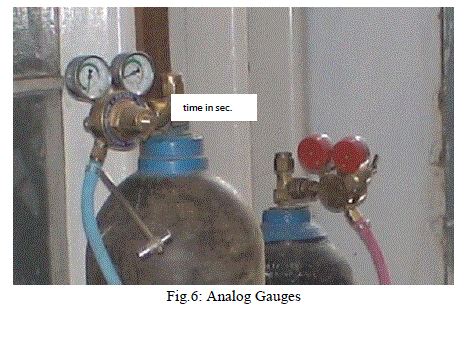 |
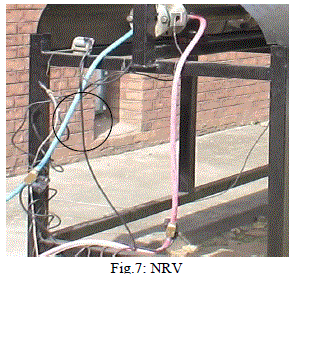 |
Figure 4b |
Figure 5a |
Figure 5b |
Figure 6 |
Figure 7 |
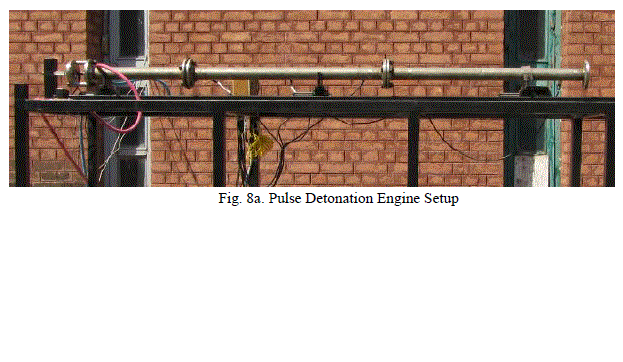 |
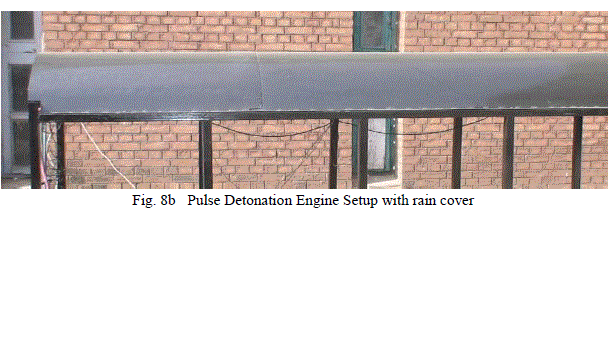 |
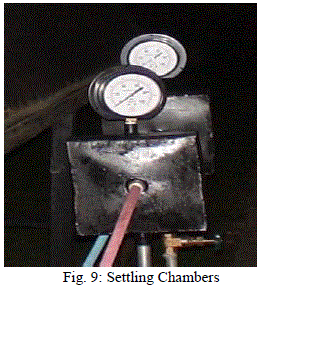 |
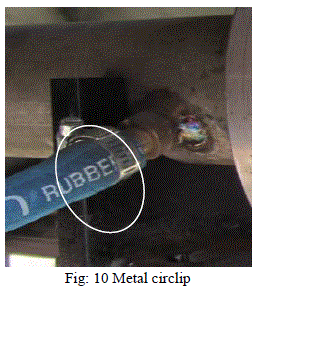 |
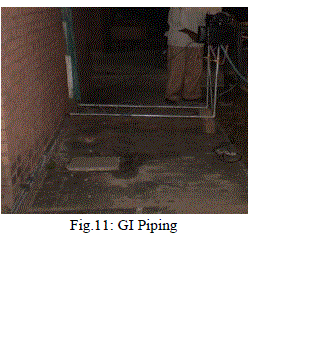 |
Figure 8a |
Figure 8b |
Figure 9 |
Figure 10 |
Figure 11 |
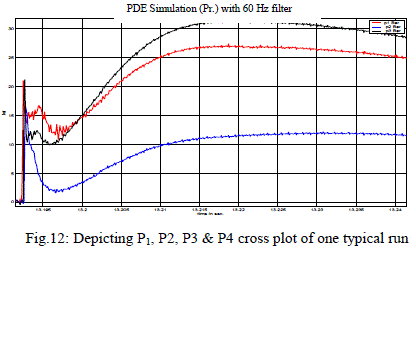 |
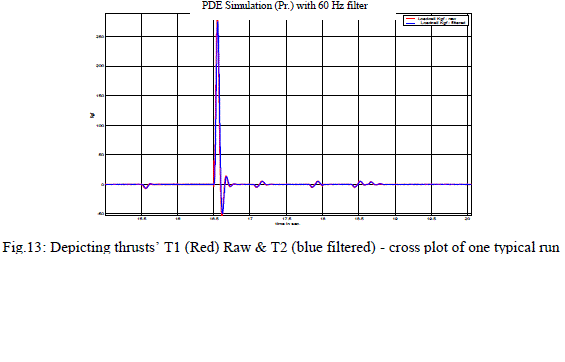 |
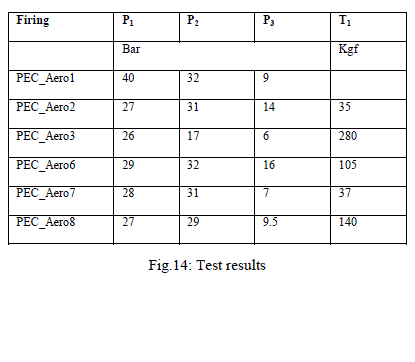 |
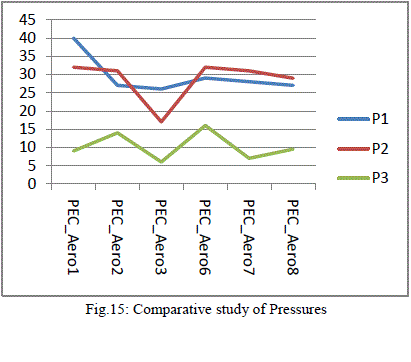 |
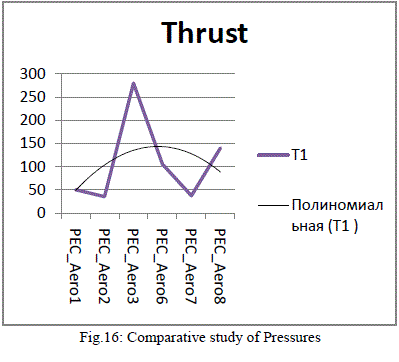 |
Figure 12 |
Figure 13 |
Figure 14 |
Figure 15 |
Figure 16 |
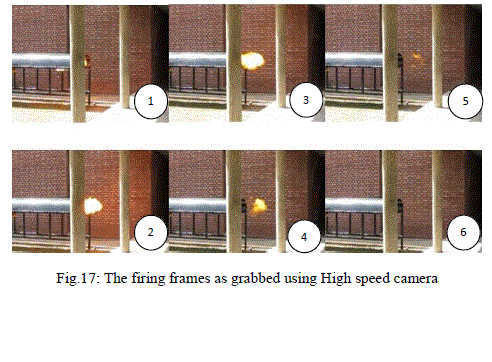 |
Figure 17 |
|
References
|
- TK Jindal, YS Chauhan, “Development of Pulse Detonation Rig”, A Report on sponsored research by TBRL, June, 2012
- H. Hoffmann, “Reaction-Propulsion Produced by Intermittent Detonative Combustion,” German Research Institute for Gliding, Report ATI-52365,August 1940.
- P.D. Hutcheson, “Design, Modelling and Performance of a Split Path Jp-10/ Air Pulse Detonation Engine”, Master?s Thesis, Naval Postgraduate School,Monterey, California, December 2006.
- P.G. Harris, S.M. Guzik, and R.A. Stowe, “Design Methodology for a Pulse Detonation Engine as a Ramjet Replacement,” AIAA Paper 2004-3400, JointPropulsion Conference and Exhibit, Ft. Lauderdale, Florida, 11-14 July 2004.
- K.K. Kuo, Principles of Combustion, Second Edition, John Wiley and Sons, 2005.
- W.H. Heiser, D.T. Pratt, “Thermodynamic Cycle Analysis of Pulse Detonation Engines,” Journal of Propulsion and Power, Vol. 18, No. 5, 2002.
- C.M Brophy, R. K. Hanson, “Fuel Distribution Effects on Pulse Detonation Engine Operation and Performance,” Journal of Propulsion and Power, Vol.22, No. 6, 2006.
- Cesium-based Velocimeter for Model Validation, Retrieved October 10, 2007, from http://hanson.stanford.edu/ research/propulsion/cesiumV.html.
- Glassman, I., Combustion, Second Edition, Academic Press, Inc., 1987.
- B.T. Channell, “Evaluation and Selection of an Efficient Fuel/Air Initiation Strategy for Pulse Detonation Engines”, Master?s Thesis, Naval PostgraduateSchool, Monterey, California, September 2005.
- G. Roy, S. Frolov, D. Netzer, A. Borisov, High-Speed Deflagration and Detonation, ELEX-KM Publishing, 2001.
- J.B. Liu, P. Ronney, and M. Gundersen, “Corona Discharge Ignition of Premixed Flames”, http://carambola.usc.edu/ research/coronaignition/coronaignition.html, September 2005.
- E. J. Touse, “Transmission of a Detonation Wave Across a Sudden Expansion with varying Mixture Composition,” Master?s Thesis, Naval PostgraduateSchool, Monterey, California, December 2003. 95
- E. Schultz, “Detonation Diffraction Through an Abrupt Area Expansion,” Doctoral Thesis, California Institute of Technology, Pasadena, California, April2000.
- C.N. Hackard, “Ignition Delay Characteristics for Transient Plasma Ignition of Acetylene/Air and JP-10/Air Mixtures in a Pulse Detonation Engine,”Master?s Thesis, Naval Postgraduate School, Monterey, California, December 2007.
- Operations and Maintenance Manual, Six Component, 500 Pound Pulse Detonation Measurement System, Pacific Press Co., N00244-02-C-0060, ManualNo. 110028, September, 2002.
- W.T. Thomson, M.D. Dahleh, Theory of Vibration with Applications, Fifth Edition, Prentice Hall, Inc., 1998. 96
- www.wikipedia.com
- Pulse Detonation Engine Technology: An Overview by Matthew Lam, Daniel Tillie, Timothy Leaver, Brian McFadden
- www.google.com
- www.nasa.com
- www.meas.com
- www.ni.com
|